Introduction
In most nations, soil contamination with heavy metals is a significant concern. These metals are naturally discovered in the earth’s crust. They have atomic numbers greater than 20 and densities greater than 5 g/cm3. The most frequent poisonous metals found in soil and water bodies are arsenic (As), mercury (Hg), cadmium (Cd), lead (Pb), chromium (Cr), nickel (Ni) and zinc (Zn). The Environmental Protection Agency (EPA) has classified all substances (except Zn) as environmental contaminants [
1-
4]. Heavy metals occur naturally in the soil due to volcanic eruption, rock weathering, and erosion. The primary natural source of heavy metals is the parent material during weathering. Nevertheless, only traces (<1000 mg/kg) of heavy metals are produced this way, which are not harmful.
However, man-made sources, including mining, energy and fuel production, military activities, municipal waste, power transmission, industrial byproducts, fertilizers, urban effluents, vehicle exhausts, smelting, and melting operations, contaminate water and soil [
5-6]. More than 140000 chemicals and pesticides have been produced since 1950, out of which 2500 are” for better understanding the meaning of sentence widely dispersed around the globe and have never been tested for their toxicological profile [
7]. Apart from the aforementioned former contaminants, contemporary carbon-based nano-materials such as carbon nanotubes, graphene, metals, and metal oxide nanoparticles also have an adverse effect on the environment. Due to their nano size, these chemicals diffuse into fog, rain, and snow after transport to the marine ecosystem by continuous cycling of volatilization and condensation. They have detrimental impacts on every life on earth, a global issue. Environmental pollution accounted for one in every nine deaths in 2012, making environmental pollution the most alarming problem and health risk to humans [
8]. The existence of heavy metals in everyday meals, such as fresh vegetables and fruits, poses a great threat to health and causes cancers, immune system imbalance, neurological problems, pulmonary irritation, heart and kidney dysfunctions, and even death due to bioaccumulation and biomagnification [
9]. In most nations, heavy metals are the primary pollutants in various ecosystems. According to estimates, nearly 80% of global pollution is subjected to the danger of water security, and in most threatening cases, the underlying cause is water pollution by heavy metals [
8]. Over 12000 industrial facilities in the Faridabad District of Haryana, India, discharge nearly 16000 kilo litres of treated wastewater into the Yamuna River every day. However, even after receiving treatment, large amounts of Cd, Zn, Ni and Cr are discovered in the river downstream, with hexavalent Cr (VI) present at a quantity greater than 600 times that advised by the World Health Organization (WHO) [
10]. There were higher blood levels of Pb, Ni, Cr and Mn than recommended by WHO in pregnant women and children in Nigeria. Also, in South Africa, the concentration of heavy metals in maternal and umbilical cord blood showed intolerably higher concentrations of Hg, Cd, Pb and Se, indicating high risks of heavy metals to adults and fetuses.
Similarly, it has been discovered that heavy metal contamination affects one-sixth of China’s total agricultural land area. In 16.1% of farming soils, the heavy metal content surpasses China’s obligations for soil environmental quality. Regarding the percentage of soil samples exceeding China’s standard limit (7.0%), Cd is the most dangerous heavy metal and metalloid. Soil pollution is also a major issue in Europe, with 3000000 potentially polluted sites, of which 250000 are highly contaminated and need to be cleaned up. In the USA, 350000 people are estimated to require remediation in the next 10 years [
7]. In nations such as Slovakia, Hungary, Sweden, France, and Austria, there is a comparatively reduced quantity of 200000 polluted sites. Greece and Poland reported only 10000 contaminated land areas, while Ireland and Portugal reported less than 10000 contaminated sites. On an approximate basis, it has been shown that 600000 hectares of Brownfield areas in America are heavily contaminated with metals [
11].
Several biological, physical, and chemical techniques have been employed for soil remediation over the past 20 years. General methods for removing toxic metals from wastewater include coagulation, precipitation, co-precipitation, electrolytic reduction, membrane filtration, ion exchange, and sorption. However, most physicochemical methods are expensive and may also prove hazardous to the environment. Evidence suggests other evolutionary techniques for eliminating these environmental pollutants, such as bioremediation, which is a natural procedure involving organisms or their products to eliminate or decrease the toxic contaminants in the environment.
Materials and Methods
The present study aimed to screen available literature and comprehensively review different globally accepted scientific databases, including Science Direct, PubMed, SpringerLink and Google Scholar. We intended to find different methodologies for the remediation of environmental pollutants, especially heavy metals.
Bioremediation
Bioremediation was introduced in 1928 when Gray and Thronton discovered that microorganisms could naturally degrade benzene, toluene, ethylbenzene and xylene (BTEX) in soil [
7]. Bioremediation generally includes natural attenuation, bioaugmentation, or bio-stimulation with a deliberate attempt to add natural or engineered microorganisms to enhance the desired catalytic capabilities [
12]. The eco-friendly agents of bioremediation include microorganisms, enzymes or plants. Among microorganisms, bacteria, algae, protozoa, fungi, and engineered microorganisms have been reported to be important in remediating soil and aquatic environments [
13]. The earliest reports of a bacterium that can degrade BTEX were published by Williams and Murray in 1974, and it was only recently revealed that microbial interactions in the rhizosphere are involved in the decontamination process [
14]. Later, it was described that one of the strains of Pseudomonas putida carrying the toluene/xylene-catabolic (TOL) plasmid used them as carbon sources and possessed an enzymatic route to degrade these compounds. Since then, different bacterial species have been found that degrade a wide range of these compounds. The most studied members belong to the genera Enterobacter, Flavobacterium, Corynebacterium, Rhodococcus, Methylosinus, Mycobacterium, Pseudomonas, Bacillus, Arthrobacter, Alcanivorax, Burkholderia, Micrococcus, Streptomyces, Sphingomonas, Cellulomonas, Marinobacter, Haemophilus, Xanthomonas, Acinetobacter, etc. These genera can decontaminate pesticides, polycyclic aromatic hydrocarbons (PAHs), and azo dyes, removing heavy metals or changing their redox state [
7,
15]. For successful bioremediation by bacteria, it is necessary to characterize the composition of the microbial community, their cellular processes, and metabolic activity in the presence of stress-induced contaminants that alter their typical behavior. The problem of incomplete information regarding cellular pathways, enzymes and encoded genes among many microbial communities can be solved by genome sequencing. According to a survey, the genomes of 270567 organisms have been sequenced. As far as bioremediation is concerned, the genomes of several microorganisms have been sequenced, like that of Pseudomonas putida KT2440 has revealed genes encoding many enzymes or proteins that show significant potential in degrading chemicals released from industrial effluents. As far as heavy metals are concerned, they cannot be degraded as such, and their bioremediation mainly involves adsorption into the cell wall, compartmentalization in organelles or vacuoles of eukaryotes, or altering their redox state to less soluble forms, thereby reducing their bioavailability. Usually, plants are the best options for such agents; a striking example is using anaerobe Geobacter sulfurreducens. In this system, acetate is pumped into the ground that is polluted by heavy metals (manganese (Mn), uranium (U), or Cr) so that G. sulfurreducens can respire acetate and transfer electrons to metals, making them unavailable by converting them into less soluble forms. The prominent feature of this process is the generation of electric current and its conduction through specialized pili, so this system is an application for bioenergy-generating alternatives.
Fungi are also useful for bioremediation, especially Basidiomycota, commonly called white-rot fungi, as they produce large quantities of extracellular oxidative and hydrolytic enzymes. Other studied genera include Trametes, Phanerochaete, Coriolopsis (Basidiomycota), Pleurotus, Bjerkandera and several different Trichoderma, Aspergillus and Fusarium (Ascomycota). Although limited reports exist on yeasts (Rhodotorula spp., Candida spp. and Yarrowia spp.), they are likely to produce enzymes like oxidases, laccases, peroxidases, intracellular monooxygenase cytochrome P450 and lytic polysaccharide monooxygenases. Their activity is quite unspecific and can use large amounts of substrates of different compounds such as pesticides, endocrine disruptors, PAHs, dyes, and even explosives such as trinitrotoluene. It should be noted that fungi grow fast and can be placed in large filters. Their oxidases, particularly laccases and peroxidases, possess a larger redox potential than bacteria and prove more efficient in the degradation of pollutants [
7]. Microalgae and some cyanobacterial species are promising bioresources, especially Anabaena, Spirogyra, Oscillatoria and Phormidium, favorably tolerating heavy metals like As, Cd, Cr, Pb and Hg. Microalgae species use multiple strategies as a protection mechanism against the toxicity of heavy metals, namely, metal immobilization, chelation, gene regulation, and redox reactions by using antioxidant and reducing enzymes [
16]. Generally, all of these methods have drawbacks, including expensive processing costs, labor-intensive, specificity, disruption of the natural soil microbiota, and permanent changes to the physicochemical properties of the soil. Bioremediation employs either ex situ or in situ techniques to remediate pollutants. The contaminated material is either shifted to another place and remediated or is treated at the original contamination site, leading to topsoil degradation and, hence, soil fertility is lost. Moreover, the biological agents secrete various enzymes and chemicals that alter the soil pH, impairing soil quality. In the Borhola oil fields in Assam, India, laboratory and field pilot research were conducted on the bioremediation of soil contaminated with petroleum hydrocarbons. Researchers looked into how the bioremediation process was affected by aeration, nutrients (such as nitrogen and phosphorus) and the inoculation of exogenous microbial consortia. The positive effects of these parameters on the bioremediation rate are seen in lab and field pilot testing. Field testing results show that up to 75% of the hydrocarbon contaminants might be broken down in one year [
17].
Phytoremediation
The green and clean technologies known as phytoremediation are receiving more attention among the many methods used to restore heavy metal-contaminated soils in situ. The term phytoremediation consists of the Greek word phyto, which means plant and the Latin word remedium, which means to restore or cure [
6,
8]. The process of using naturally occurring or genetically modified plants to remove dangerous materials from the environment, such as pesticides, radio nucleosides, polychlorinated biphenyls, and polynuclear aromatic hydrocarbons and then break them down and transform them into safe metabolites, is known as phytoremediation. Additionally, phytoremediation can remediate not only metals but also other contaminants, including explosive components, petroleum hydrocarbons, PAHs, surfactants, and chlorinated solvents. In 1983, Chaney introduced this concept, but for the past 300 years, this notion has already been ingrained. Later on, several plant species were discovered that possess the capacity to eliminate hazardous metals from contaminated surroundings. Several terms, including green remediation, vegetative remediation, agro-remediation, green technology, and botano-remediation, are used and preferred to phytoremediation. Phytoremediation is a highly effective green technology for accumulating various heavy metals. It involves using soil, plants, and bacteria with other agrochemical practices. Both in situ and ex situ remediation are useful in a phytoremediation procedure. The in situ approach is employed more frequently because it minimizes the harm to the surrounding environment by reducing the multiplication of contaminants in water and airborne debris at an early stage. This technique allows many types of pollutants to be treated on-site without needing a disposal location.
Later on, several plant species were discovered that can detoxify toxic substances from contaminated surroundings. Additional advantages include decreasing the spread of contamination by stopping soil erosion and leaching. The utmost advantage of this technique is that the clean-up cost is far less than other conventional remediation techniques. Phytoremediation is a relatively simple procedure because it does not require specialized staff or expensive equipment. Where other traditional procedures prove exceedingly inefficient and not economically possible, large-scale areas can be subjected to phytoremediation. From an economic perspective, phytoremediation has three levels of goals: 1) Plant-based metal extraction with financial gain, 2) Risk reduction, also known as photo-stabilization, and 3) Sustainable soil management, whereby phytoremediation progressively raises soil fertility with additional economic benefit. Many plant species can generate financial benefits. Many plant species can accumulate much higher quantities of heavy metals in different plant areas, like the leaf, stem, and root, without exhibiting any harmful effects. To make phytoremediation an environmentally sustainable technology, plants must possess certain typical traits such as fast growth rates, high biomass yields, the ability to absorb large amounts of heavy metals, transport metals to the upper parts of the plant, and the ability to withstand metal toxicity. The plant growth that produces high biomass also depends on other elements, including salinity, pH, sunshine, and nutrient requirements. Phytoremediation trials were conducted in a former U mining region in East Germany. The test field site exhibited mild to moderate heavy metal/radionuclide (HM/R) contamination. A mix of phytostabilization and phytoextraction strategies were employed, utilizing microbial, soil, and plant techniques at field and lab scales. To learn more about how soil amendment techniques (fertilizing, raising pH and organic matter, adding fungus and bacteria) affect plant tolerance to heavy metals and biomass production, plant experiments were conducted using C, Helianthus annuus and Brassica juncea. Reduced seepage water rate and load, groundwater contamination, and HM/R buildup in soil could all be demonstrated in lysimeter studies. Following harvests, the ultimate use of HM/R-loaded plant leftovers was investigated using ethanolic and biogas fermentation and plant material combustion. The investigation focused on the fate of HM/R in the various byproducts [
18]. Numerous quickly growing tree species, such as poplar, jatropha and willow, are being used for their ability to produce energy and act as phytoremediators.
Around 24 plant species, divided into 16 families, were discovered in the Alacran mine, where Hg concentrations in the soil ranged from 230 to 6320 ng/g. Of these, the highest concentrations of Hg were found in the roots and shoots of Jatropha curcas, Piper marginatum, Capsicum annum, Thalia geniculata, Cyperus ferax and Sticherus bifidus. J. curcas, in particular, was discovered to have the highest Hg bioaccumulation (5983 ng/g in roots and a bioaccumulation factor of 0.99), suggesting that artisanal and small-scale gold mining operations may be able to use this plant as a remedy for Hg-contaminated soils [
19]. The sustainable phytoremediation technology is based on different uptake mechanisms, including phytoextraction, phytostabilization, phytoevaporation, rhizofiltration, and rhizodegradation.
Phytoextraction
Phytoextraction refers to the absorption of metals by plants and associated soil microbes to reduce environmental pollutants’ levels or toxic effects [
5]. Since harvesting the root biomass is usually impractical, metal translocation to aboveground portions like shoots is a desirable biochemical process for effective phytoextraction. Once the phytoextraction is over, the plant can be harvested and burned to gain energy, and metal can be recovered from the ash, if required. Phytoextraction presents a more difficult task than other forms of phytoremediation because harmful contaminants are found in larger concentrations in soil. Plants should take up metals from the soil, move them, and concentrate them in the sections of the plant above ground. The plants employed in phytoextraction must withstand metals and develop quickly enough to yield a large amount of biomass. However, most plants that accumulate metals grow slowly and produce little biomass, which has caused the phytoextraction process to proceed very slowly. The development of phytoextraction technology has been greatly aided by discovering hyperaccumulators, plants that can absorb heavy metals at concentrations 50-500 times higher than typical plants. Baker and Brooks believed hyperaccumulator plants could collect >10000 mg/kg of Mn and Zn, 1000 mg/kg of As, Co, Cu, Pb, Ni, or 100 mg/kg of Cd. The digestion and passage of metals via root cell membranes, loading into the xylem, transferring to shoots, and sequestering and detoxifying metals within plant tissues are some of the processes that lead to the hyperaccumulation of heavy metals. The epidermis, cuticle, and trichomes are the best places for metal detoxification. About 400 plants have been known as hyperaccumulators, which comprise only <0.2% of higher plants, belonging to families like Brassicaceae, Asteraceae, Fabaceae, Lamiaceae, Caryophyllaceae, Poaceae, Cyperaceae, Cunoniaceae, Flacourtiaceae, Euphorbiaceous, Scrophulariaceae and Violaceae [
11,
20, 21]. Over 320 species of Ni hyperaccumulators, 34 species of Cu, 30 species of Co, 20 species of Se, 14 species of Pb, 11 species of Zn, 10 species of Ni, 4 species of As and 1 species of Cd are known to exist. Several plant species are known to accumulate heavy metals, including Astragalus racemosus, Viola calaminaria, B. juncea, and Alyssum spp. [
21]. To a large degree, however, the ability to hyperaccumulate metals is a defining trait of the Brassicaceae family. The common heavy metals it scavenges are Pb, Cd, Zn and Ni. There are 87 species. and 11 genera in this family; of these, 72 species and 7 genera accumulate Ni, 15 species and 4 genera accumulate Zn. Sebertia acuminata, a tiny tree from New Caledonia, is the best hyperaccumulator of Ni, with 25% of Ni in its dried bluish-green latex. Various species of Thlaspi can accumulate multiple metals. Thlaspi caerulescens accumulates Cd, Ni, Pb and Zn, Thlaspi rotundifolium accumulates Ni, Pb, and Zn, and Thlaspi goesingense and Thlaspi ochroleucum accumulate Ni and Zn. According to reports, B. juncea tissues contain one-third of the concentration of Zn, making them more effective at remediating Zn than T. caerulescens, a generally recognized Zn hyperaccumulator. The fundamental cause of this phenomenon is that B. juncea produces ten times as much biomass as T. caerulescens. Through experimental research, the capacity of many Brassica species to withstand and accumulate harmful metals has been confirmed. The primary plant for removing heavy metals from the soil, such as Cd, Pb, Cr (IV), Cs, Cu, Ni, U, and Zn, is Indian mustard (B. juncea). Salix spp. and Populus spp. are largely used for Cd and Zn extraction from moderately contaminated soils. Pteris vittata, a fern, is the first hyperaccumulator for As as per reports and removed 70% of As in 3 years which was highest among four As accumulating species including Adiantum capillus-veneris (60%), Phragmites karka (56.1%), Christella dentata (55.1%), and P. vittata [
22-
30]. Various other species of fern identified as potential As hyperaccumulators include Dryopteris filix-mas, Pteridium aquilinum, Pityrogramma calomelanos, Pteris cretica, Pteris longifolia, and Pteris umbrosa. Among the herbs Blumea lacera, Mimosa pudica, Mikania cordata and Ageratum conyzoides and among the shrubs, Melastoma malabathricum, Clerodendrum trichotomum and Ricinus communis also seemed to have potential for phytoremediation of As contaminated soils [
21,
31-
36]. According to Jankong et al. [
32] study, using phosphorus fertilizer and rhizosphere bacteria on silverback fern, P. calomelanos, significantly enhanced As-phytoextraction. Similarly, Souza et al. [
37] demonstrated that Lemna valdiviana absorbed a greater amount of As at a pH of 6.3-7, phosphorus availability of 0.0488 mmol/L, and nitrogen (as nitrate) at a concentration of 7.9 mmol/L. A few floating plants, including Azolla pinnata, Eichhornia crassipes, Spirodela polyrhiza, Chlorodesmis spp., Cladophora spp. and a wetland weed called Monochoria vaginalis, can also prove promising members for As+3 contaminated surface water and wetlands [
34,
38]. There are many types of hyperaccumulators for the phytoremediation procedure currently in trend, including A. racemosus, Zea mays and Eichhornia crassipes (water hyacinth), the latter being the most idealistic oceanic plant [
11]. The water hyacinth has proved to be a powerful candidate in the phytoremediation of poisons like Cd, Co, As, Ni, Hg and Pb from antiquated times to the present era [
39]. In addition to water hyacinth, other well-known metal accumulator plants for cleaning up heavy metal-polluted water include water lettuce (Pistia stratiotes), duckweed (Lemna minor) and a few other aquatic plants [
40]. Several other plant species, including sunflower (H. annuus), remediate radionuclides from the polluted soil. The advantages that plants possess due to hyperaccumulation and their detailed mechanisms remain unclear. However, most data on Ni hyperaccumulation mechanisms has been found for the Thlaspi and Alyssum genera (Brassicaceae) and the South African species of Berkheya coddii (Asteraceae). B. coddii has a higher biomass than other hyperaccumulator plants and can successfully absorb and translocate Ni from the plant roots to the aboveground shoots. It is highly tolerant to Ni in the soil and the tissues. Metal binding peptides have been synthesized using biotechnological techniques and genetic engineering tools to boost hyperaccumulator plants’ biomass and growth rate. The initial scientific breakthroughs were reported using Populus for cleaning up soil contaminated with Cd and Cu, Populus balsamifera for cleaning up As-contaminated soil, Liriodendron tulipifera and Arabidopsis thaliana for cleaning up Hg, and Nicotiana tabacum for cleaning up Cd [
11,
40]. Nicotiana glauca, which has been genetically engineered, has shown promise in soil restoration. With 100 times larger biomass, the T. caerulescens hyperaccumulator possesses a higher concentration of heavy metals than the transgenic N. glauca. The use of chelating compounds such as ethylenediaminetetraacetic acid (EDTA), ethylenediamine dihydroxy phenylacetic acid and hydroxyethyl ethylenediaminetetraacetic acid (HEDTA) is beneficial when the availability of heavy metals in the soils is insufficient for the active root absorption. Such compounds improve Cu, Cd, Pb, Zn and Ni phytoextraction. Chelation, however, has many drawbacks, including harmful effects on soil microbes, groundwater contamination, and a sluggish rate of synthetic organic acid breakdown. Rapidly degrading natural organic acids such as citric acid and oxalic acids, tartaric acid, malic acid, and putrescine (polyamine) can overcome these limitations. More investigation is needed on using these substances to improve plant uptake of metals and the disadvantages of their removal while considering the rate of their biodegradation. Therefore, to minimize the residual effects of metal chelates on soil microorganisms, it is important to carefully choose the chelating agents, use an appropriate dosing strategy and apply the treatment at the appropriate time. In most cases, phytoextraction took longer (up to tens of years) to remediate soil polluted with heavy metals. In France, the Cd concentration can be lowered by 10 μg/kg in a single season by employing T. caerulescens for phytoextraction of polluted soil. It will never be possible to reduce the amount of Zn in the soil from high to low levels of biological accumulation in an economically profitable way (less than 10 years). It took two years for B. coddii to restore moderately Ni-polluted (100 mg/kg) soils in New Zealand to a standard concentration typically accepted by the EU (75 mg/kg). It would take 35-139 years for highly polluted soils (2000-10000 mg/kg) with 23 t/ha biomass. A 3-year study conducted in a greenhouse with various plant species found that the overall level of Zn decreased by 50% and the total content of Cd by 20%. Therefore, the phytoextraction process has the same benefits and limitations as clean-up [
11]. Various plant species have been found to remediate heavy metals (
Table 1).
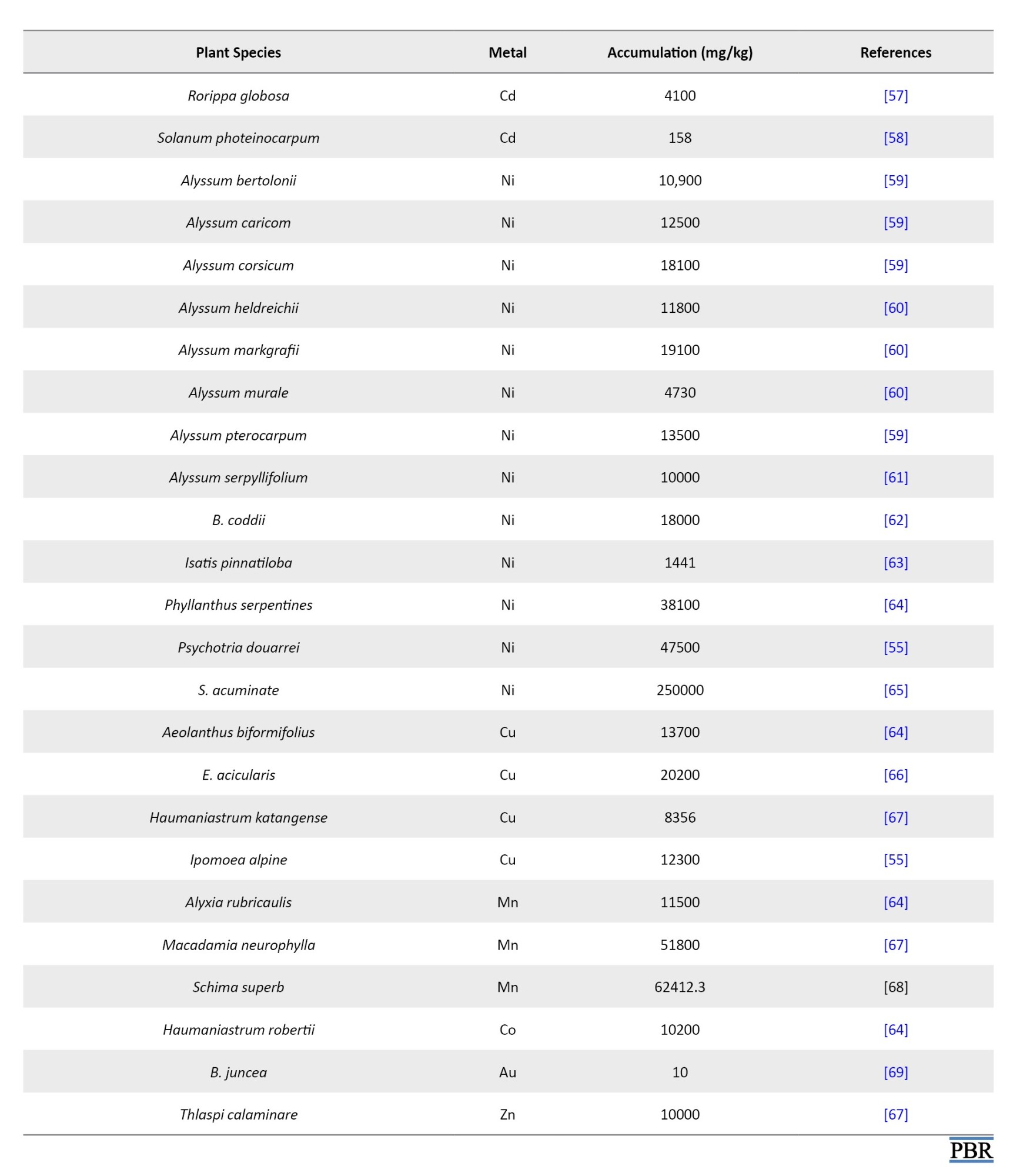
When determining how long the phytoextraction process will take, it is crucial to consider how changes in the concentration of heavy metals in soil would affect plant adsorption during the phytoremediation process. The reduction of heavy metal contents in soil during phytoextraction may cause plants to absorb lower heavy metals, extending the time needed for phytoextraction to achieve the initial target levels. If the outcomes of these brief trials are extrapolated with constant plant uptake rates, the length of phytoextraction may be underestimated [
70]. A field cultivation experiment was performed in Japan to study the suitability of the macrophyte Eleocharis acicularis as a hyperaccumulator. It was found that E. acicularis has the potential for phytoremediation of soils containing high amounts of Zn, Pb, As, Cu and Cd. It can be used for phytoextraction in humid regions of Asia with the same climate and soils as the mentioned study site [
66].
Phytostabilization
Phytostabilization is the process in which plants help reduce the mobility and bioavailability of heavy metals in soils due to their stabilization from off-site transport. Phytostabilization uses plants’ ability to accumulate specific heavy metals in their root zone through phytosequestration or phytodeposition [
11]. The primary conditions necessary for the plant species to be used for phytostabilization include an extensive root system, rapid growth rate, resistance to heavy metals, and a poor translocation of metals from roots to shoots. The phytostabilization technique aims to reduce the risk of heavy metal pollution by stabilizing them through the development of a vegetative cap at the plant rhizosphere, where the sequestration (binding and sorption) process immobilizes metals to minimize the threat and make them unavailable to livestock, wildlife and human exposure. Various organic and inorganic soil amendments that encourage precipitation and the absorption of metals can be done to enhance the fixation. Phytostabilization is economically feasible and easy to execute; therefore, it is more advantageous than other techniques. This technique is applied to a wide range of surface contamination sites and sites with high organic loads and porosity. However, this technique has a significant drawback: It does not apply to heavily contaminated areas because such conditions hinder plants’ growth rate. Moreover, this technique is not a permanent resolution as it does not eliminate the metals from the soil but only restricts their movement in the soil. Phytostabilization reduces the amount of water passing through the soil, which leads to stabilization.
Because of possible effects on the food chain, metals easily translocated to plant leaves may limit the applicability of phytostabilization. This technique is employed generally to remediate soils contaminated mainly with As, Zn, Pb, Cd, Cu and Cr. The species that accumulate heavy metals in their root systems and inhibit the release of heavy metals into the food chain are the most effective hyperaccumulators for phytostabilization. The technique has flourished in recent years, predominantly for commercially available agricultural grasses. Certain plant species like Agrostis spp. and Festuca spp. are commonly used in the phytostabilization of soils contaminated with Cu, Pb and Zn in Europe, where they have originated, and in America and China, where they were introduced later.
In some cases, serious issues may be raised, particularly when the soil is heavily contaminated and degraded. In such conditions, it is advantageous to use the routine plant species adapted to the local environment and tolerant to metal contamination, along with adding fertilizers and liming. Members of the Chenopodiaceae family, especially Atriplex spp. are utilized to revegetate mine tailings in the Western United States and act as pioneer species in semiarid western Australia. They are also highly salt tolerant [
71].
Phytovolatilization
Phytovolatilization, or photoevaporation, is when toxic heavy metals like Hg, Se, and As are transformed into less hazardous and volatile forms and released into the atmosphere [
11]. This technology is generally employed to remediate soil, groundwater, sludges, and sediments [
40]. Since Se is a sulfur analog, plant enzymes involved in sulfur metabolic pathways, absorption, and volatilization transform Se to dimethyl selenide [
72]. Certain species of the Brassicaceae family, such as B. juncea, assimilate Se from the soil and convert it into organic seleno-amino acids, seleno-methionine, and selenocysteine, which then get volatilized into the atmosphere. Its wild species cause phytovolatilization of Hg in roots [
72]. Although this remediation strategy provides certain benefits, such as less site disturbance and erosion, it is still regarded as the most contentious and restricted of all phytoremediation technologies because this process does not fix the contaminants completely but transfers pollutants from soil to the atmosphere from where they can be redeposited. For example, when Hg is released into the atmosphere, it will likely be recycled by precipitation and redeposited into the ecosystem. Since high levels of Hg and Se are poisonous, it is debatable whether these metals are safe or desirable to volatilize into the atmosphere. The problem was solved by a transgenic plant showing excellent Hg volatilization and it was possible to incorporate the Hg ion reductase gene of bacteria in A. thaliana. Additionally, it has been observed that when model plants like N. tabacum and A. thaliana were modified to include the bacterial mercuric reductase (merA) and organomercurial lyase (merB) genes, the resultant transgenic plants can absorb elemental and methyl Hg from the soil for volatilization [
73]. Compared to non-transgenic plantlets, transgenic yellow poplar (Liriodendron tulipifera) plantlets volatilized about 10 times more elemental Hg and exhibited resistance to toxic concentrations of the metal. This technology is currently employed to decay tritium (H-3), a radioactive isotope of hydrogen, into stable helium with a half-life of about 12 years [
6,
11].
North Island, New Zealand’s Tui mining tailings were the site of research. The study’s main objective was to investigate Hg uptake into aboveground plant tissues through the solubilization of Hg in Tui mine tailings produced by thiosulphate. In field-grown B. juncea plants, applying sodium thiosulfate (Na2S2O3) successfully stimulated Hg uptake by roots and translocation by shoot. The highest yield of extraction was around 25 g Hg/ha. Studies on volatilization showed that plants significantly accelerated the transformation of Hg in Tui tailings to Hg (0). According to mass balance estimates, Hg volatilization eliminated about 500 g Hg/ha over the trial period. About 95% of the total Hg mass extracted from the Tui mine tailings is represented by this value [
74].
Rhizofiltration
Rhizofiltration or phytofiltration is “a form of phytoremediation in which plant uses its roots to absorb, concentrate, and precipitate the contaminants present in the groundwater, thereby causing restriction in movement of these contaminants in underground water.” Plant roots act as filters to adsorb pollutants [
21]. Plants for rhizofiltration should have characteristic features: Extensive root system, a tendency to accumulate large amounts of heavy metals, ease of handling, and low maintenance cost. Rhizofiltration is applied effectively to treat heavy metals, radioactive pollutants, agricultural runoff, and industrial discharge. However, terrestrial plants are the best options for rhizofiltration as they possess a strong and fibrous root systems and a greater capacity to absorb metals [
75]. Indian mustard (B. juncea L.) and sunflower (H. annuus L.) are the main examples of Indian mustard, which is known to remove a large concentration of Pb (4–500 mg/L). Rhizofiltration is generally used for metals such as Pb, Cu, Zn, Cd, Ni, Cr and As retained within the roots. A few examples of plants applicable for rhizofiltration include sunflower, Indian mustard, tobacco, rye, corn, and spinach, with sunflower having the most remarkable ability, and that too for Pb [
76].
Blastofiltration (blasto means “seedling” in Greek) is another advanced technique in which young seedlings in water help remove heavy metals. Young seedlings are ideal for repairing water quality because, in this technique, the surface-to-volume ratio improves significantly after germination. Some germinating seedlings also adsorb large amounts of hazardous metal ions. Blastofiltration is more active and cost-effective than rhizofiltration, but rhizofiltration has an advantage over blastofiltration in its ability to be employed in situ and ex situ [
6]. The only ingredients for blastofiltration seedling cultures are seeds, water, and air. These cultures can be grown in either light or dark conditions [
77]. At a former U-processing factory in Ashtabula, OH, rhizofiltration technology has been tested in the field with U-contaminated water at 21−874 μg/L concentrations. Before being released into the environment, the site source water was finally treated by the pilot-scale rhizofiltration system using B. juncea and H. annuus, bringing the U concentration down to less than 20 μg/L (EPA water quality standard). Over 200000 L of wastewater was treated using sunflower plant-based rhizofiltration technology [
78].
Rhizodegradation
Rhizodegradation is the process of the breakdown of soil pollutants by the action of microbes in the rhizosphere (i.e. the zone rich in microbes that are closely connected to the plant’s vascular root system). It is considered a method of purification of contaminated water bodies due to plant roots’ absorption, concentration, and precipitation of pollutants, especially organic ones and some metals. It is due to the intricate ecosystems of plants and microbes that function as biogeochemical filters and can successfully eliminate the contaminants from sewage. Constructed waterlogged areas are beneficial for the filtration of large sewage volumes. In contrast, expensive laboratory hydroponic installations are being used to remediate hazardous inorganic compounds, including heavy metals and radionuclides, for relatively small volumes of sewage. Carex pendula, an important perennial herbaceous plant growing in moist habitats, decontaminates soil containing Pb under in situ conditions and can tolerate a high (1600 mg/kg) amount of Pb at a concentration of 10 mg/L in water [
11].
Role of microbes in enhancing phytoremediation
The plant growth-promoting rhizobacteria (PGPR) can improve the efficacy of phytoremediation by fostering plant growth, improving plants’ uptake and translocation of heavy metals, and protecting plants against harmful pathogens. These bacteria produce various compounds such as siderophores, organic acids, antibiotics, enzyme1-aminocyclopropane-1-carboxylate (ACC) deaminase, and some phytohormones like Indole 3-acetic acid (IAA) [
79]. The ACC deaminase degrades the ethylene precursor ACC, decreasing ethylene production and promoting plant growth. IAA stimulates plant growth by forming extensive root systems and protects plants against abiotic stress [
80]. Bacteria produce siderophores, which can bind with Fe3+ with a high affinity and solubilize this metal for its effective plant uptake [
79].
Arbuscular mycorrhizal fungi (AMF) occur in most ecosystems’ soil and aid their hosts’ nutritional status by delivering micronutrients, phosphate, and water. In the same way, fungal hyphae take up heavy metals and later on transported to the plant. Thus, AMF leads to enhanced uptake of heavy metals by plants and root-to-shoot transport, thereby enhancing phytoextraction. In some cases, it causes immobilization of heavy metals within the soil, aiding in phytostabilization [
81].
Advantages and limitations of phytoremediation
Although phytoremediation is an attractive option to remediate a good range of obnoxious heavy metals, being environmentally friendly and economically feasible, there are the following limitations.
1. The technique is effective if soil contamination is within a range of 3 feet of the surface and groundwater is within 10 feet of the surface [
20].
2. Soil remediation requires a more extended time (several years).
3. It applies to sites with low to moderate contamination and sites with large volumes of groundwater with low contamination that must be cleaned to low standards [
20].
4. The phytoextraction efficacy of most metal hyperaccumulator plants is generally restricted by their low biomass and slow growth rate.
5. In tropical and sub-tropical regions that are affected by climate, the potential of heavy metal accumulation by plants is weakened and ineffective due to diseases and pest attacks.
6. Because of the restricted bioavailability of the pollutants in the soil, it is difficult to mobilize a more tightly bound proportion of heavy metal ions from the soil.
7. The introduction of alien plant species with the potential of hyperaccumulation is generally avoided due to its deleterious impact on native floral diversity.
8. The mobility of metal ions in soil may be adversely affected by various agronomic techniques and amendments done in soil.
9. Climatic and weather conditions are the primary determinants of sustainable phytoremediation.
10. Inappropriate biomass handling increases the possibility of accumulated metals entering the food chain.
Conclusion
Heavy metal pollution threatens soil and water quality and food security, especially because of their non-biodegradable nature and bioaccumulation in the food chain. Conventional methods to remedy this problem were ineffective due to their high cost, soil quality, soil microflora destruction, and production of secondary pollutants. Phytoremediation is an alternative and sustainable approach to countering environmental heavy metal pollution. Various plants possess inherent biological mechanisms that help them to tolerate heavy metal stress. Phytoremediation, phytoextraction, and phytostabilization are very effective techniques, and phytoextraction is expected to be a commercially important technology for phytoremediation in the future. Hyperaccumulators definitely play a significant role in metal accumulation and phytoextraction but have limitations like low biomass production, time consumption, and slow growth rate. Their use depends on the soil type and level of metal contamination. Transgenic microbes and plants can be used to remediate the contaminated sites efficiently. Understanding heavy metal uptake, translocation, and detoxification mechanisms in plants and identifying different signaling pathways is needed to design the ideal plant species for phytoremediation via genetic engineering. Using chelating agents and microbes has proven effective in increasing metal bioavailability for efficient phytoextraction and soil health for rapid plant growth. Further research is needed to understand the mechanisms of metal transport and accumulation in plants to develop better transgenic varieties for effective, economical, and time-saving phytoremediation.
Ethical Considerations
Compliance with ethical guidelines
There were no ethical considerations to be considered in this research.
Funding
This research did not receive any grant from funding agencies in the public, commercial, or non-profit sectors.
Authors' contributions
Investigation, methodology, formal analysis and writing the original draft: Pawan Kumar Verma; Resources, conceptualization, review and editing: Priyanka Shamra and Bhawani Singh; Supervision and final approval: Nrip K Pankaj and Rajinder Raina.
Conflict of interest
The authors declared no conflict of interest.
Acknowledgments
The authors are thankful to Dean, Faculty of Veterinary Sciences & Animal Husbandry, R S Pura.
References
- O'Connor D, Zheng X, Hou D, Shen Z, Li G, Miao G, et al. Phytoremediation: Climate change resilience and sustainability assessment at a coastal brownfield redevelopment. Environ Int. 2019; 130:104945. [DOI:10.1016/j.envint.2019.104945] [PMID]
- Selvi A, Rajasekar A, Theerthagiri J, Ananthaselvam A, Sathishkumar K, Madhavan J, et al. Integrated remediation processes toward heavy metal removal/recovery from various environments-a review. Front Environ Sci. 2019; 7:66. [DOI:10.3389/fenvs.2019.00066]
- Jaskulak M, Grobelak A, Grosser A, Vandenbulcke F. Gene expression, DNA damage and other stress markers in Sinapis alba L. exposed to heavy metals with special reference to sewage sludge application on contaminated sites. Ecotoxicol Environ Saf. 2019; 181:508-17. [DOI:10.1016/j.ecoenv.2019.06.025] [PMID]
- Sharma P, Pandey AK, Udayan A, Kumar S. Role of microbial community and metal-binding proteins in phytoremediation of heavy metals from industrial wastewater. Bioresour Technol. 2021; 326:124750. [DOI:10.1016/j.biortech.2021.124750] [PMID]
- Ali H, Khan E, Sajad MA. Phytoremediation of heavy metals--concepts and applications. Chemosphere. 2013; 91(7):869-81. [DOI:10.1016/j.chemosphere.2013.01.075] [PMID]
- Haq S, Bhatti AA, Dar ZA, Bhat SA. Phytoremediation of heavy metals: An eco-friendly and sustainable approach. In: Hakeem KR, Bhat RA, Qadri H, editors. Bioremediation and Biotechnology. Berlin: Springer; 2020. [DOI:10.1007/978-3-030-35691-0_10]
- Otero-Blanca A, Folch-Mallol JL, Lira-Ruan V, Carbente MDRS, Batista-Garcia, RA. Phytoremediation and fungi: An underexplored binomial. In: Prasad R, Aranda E, editors. Approaches in bioremediation. Berlin: Springer; 2018. [DOI:10.1007/978-3-030-02369-0_5]
- Dangi AK, Sharma B, Hill RT, Shukla P. Bioremediation through microbes: Systems biology and metabolic engineering approach. Crit Rev Biotechnol. 2019; 39(1):79-98. [DOI:10.1080/07388551.2018.1500997] [PMID]
- Sarker A, Kim JE, Islam ARMT, Bilal M, Rakib MRJ, Nandi R, et al. Heavy metals contamination and associated health risks in food webs-a review focuses on food safety and environmental sustainability in Bangladesh. Environ Sci Pollut Res Int. 2022; 29(3):3230-45. [DOI:10.1007/s11356-021-17153-7] [PMID] [PMCID]
- Gupta B, Mishra A, Singh R, Thakur IS. Fabrication of calcite based biocomposites for catalytic removal of heavy metals from electroplating industrial effluent. Environ Technol Innov. 2021; 21:101278. [DOI:10.1016/j.eti.2020.101278]
- Mahar A, Wang P, Ali A, Awasthi MK, Lahori AH, Wang Q, et al. Challenges and opportunities in the phytoremediation of heavy metals contaminated soils: A review. Ecotoxicol Environ Saf. 2016; 126:111-21. [DOI:10.1016/j.ecoenv.2015.12.023] [PMID]
- Shukla KP, Sharma S, Singh NK, Singh V, Bisht S, Kumar V. Rhizoremediation: A promising rhizosphere technology. In: Patil Y, editor. Applied bioremediation-Active and passive approaches. London: IntechOpen; 2013. [DOI:10.5772/56905]
- Quintella CM, Mata AMT, Lima LCP. Overview of bioremediation with technology assessment and emphasis on fungal bioremediation of oil contaminated soils. J Environ Manage. 2019; 241:156-66. [DOI:10.1016/j.jenvman.2019.04.019] [PMID]
- Shagol CC, Chauhan PS, Kim KY, Lee SM, Chung JB, Park KW, Sa TM. (2011). Exploring the potential of bacteria-assisted phytoremediation of arsenic-contaminated soils. Korean J Soil Sci Fertiliz. 44(1):58-66. [DOI:10.7745/KJSSF.2011.44.1.058]
- Verma S, Kuila A. Bioremediation of heavy metals by microbial process. Environ Technol Innov. 2019; 14:1-11. [DOI:10.1016/j.eti.2019.100369]
- Leong YK, Chang JS. Bioremediation of heavy metals using microalgae: Recent advances and mechanisms. Bioresour Technol. 2020; 303:122886. [DOI:10.1016/j.biortech.2020.122886] [PMID]
- Gogoi BK, Dutta NN, Goswami P, Mohan TK. A case study of bioremediation of petroleum-hydrocarbon contaminated soil at a crude oil spill site. Adv Environ Res. 2003; 7(4):767-82. [DOI:10.1016/S1093-0191(02)00029-1]
- Willscher S, Mirgorodsky D, Jablonski L, Ollivier D, Merten D, Buchel G, et al. Field scale phytoremediation experiments on a heavy metal and uranium contaminated site, and further utilization of the plant residues. Hydrometallurgy. 2013; 131:46-53. [DOI:10.1016/j.hydromet.2012.08.012]
- Marrugo-Madrid S, Turull M, Montes GE, Pico MV, Marrugo-Negrete JL, Diez S. Phytoremediation of mercury in soils impacted by gold mining: A case-study of Colombia. In: Saxena G, Kumar V, Shah MP, editors. Bioremediation for Environmental Sustainability. Amsterdam: Elsevier; 2021. [DOI:10.1016/B978-0-12-820524-2.00007-9]
- Ghosh M, Singh SP. A Review on Phytoremediation of heavy metals and utilization of its byproducts. Appl Ecol Environ Res. 2005; 3:1-18. [DOI:10.15666/aeer/0301_001018]
- Kaur D, Singh A, Kumar A, Gupta S. Genetic engineering approaches and applicability for the bioremediation of metalloids. In: Kumar Tripathi D, Pratap Singh V, Ramawat N, editors. Plant life under changing environment. Cambridge: Academic Press; 2020. [DOI:10.1016/B978-0-12-818204-8.00010-2]
- Ma LQ, Komar KM, Tu C, Zhang W, Cai Y, Kennelley ED. A fern that hyperaccumulates arsenic. Nature. 2001; 409(6820):579. [DOI:10.1038/35054664] [PMID]
- Fayiga AO. Phytoremediation of arsenic-contaminated soil and groundwater. [doctoral dissertation]. Gainesville: University of Florida; 2005. [Link]
- Rathinasabapathi B, Ma LQ, and Srivastava, M. Arsenic hyperaccumulating ferns and their application to phytoremediation of arsenic contaminated sites. Floriculture ornamental plant biotechnol. 2006; 3(32):304-11. [Link]
- Tong-Bin C, Xiao-Yong L, Ze-Chun H, Lei M, Wen-Xue L, Liang-Yu M, et al. Phytoremediation of arsenic-contaminated soil in China. In: Willey N, editor. Phytoremediation. Totowa: Humana Press; 2007. [DOI:10.1007/978-1-59745-098-0_26]
- Zhu YG, Rosen BP. Perspectives for genetic engineering for the phytoremediation of arsenic-contaminated environments: From imagination to reality? Curr Opin Biotechnol. 2009; 20(2):220-4. [DOI:10.1016/j.copbio.2009.02.011] [PMID]
- Ye WL, Khan MA, McGrath SP, Zhao FJ. Phytoremediation of arsenic contaminated paddy soils with Pteris vittata markedly reduces arsenic uptake by rice. Environ Pollut. 2011; 159(12):3739-43. [DOI:10.1016/j.envpol.2011.07.024] [PMID]
- Mandal A, Purakayastha TJ, Patra AK, Sanyal SK. Phytoremediation of arsenic contaminated soil by Pteris vittata L. II. Effect on arsenic uptake and rice yield. Int J Phytoremediation. 2012; 14(6):621-8. [DOI:10.1080/15226514.2011.619228] [PMID]
- Raj A, Singh N. Phytoremediation of arsenic contaminated soil by arsenic accumulators: A three year study. Bull Environ Contam Toxicol. 2015; 94(3):308-13. [DOI:10.1007/s00128-015-1486-8] [PMID]
- Fayiga AO, Saha UK. Arsenic hyperaccumulating fern: implications for remediation of arsenic contaminated soils. Geoderma. 2016; 284:132-43. [DOI:10.1016/j.geoderma.2016.09.003]
- Visoottiviseth P, Francesconi K, Sridokchan W. The potential of Thai indigenous plant species for the phytoremediation of arsenic contaminated land. Environ Pollut. 2002; 118(3):453-61. [DOI:10.1016/S0269-7491(01)00293-7] [PMID]
- Jankong P, Visoottiviseth P, Khokiattiwong S. Enhanced phytoremediation of arsenic contaminated land. Chemosphere. 2007; 68(10):1906-12. [DOI:10.1016/j.chemosphere.2007.02.061] [PMID]
- Singh N, Ma LQ. Assessing plants for phytoremediation of arsenic-contaminated soils. In: Willey N, editor. Phytoremediation. Totowa: Humana Press; 2007. [DOI:10.1007/978-1-59745-098-0_24]
- Mahmud R, Inoue N, Kasajima SY, Shaheen R. Assessment of potential indigenous plant species for the phytoremediation of arsenic-contaminated areas of Bangladesh. Int J Phytoremediation. 2008; 10(2):117-30. [DOI:10.1080/15226510801913884] [PMID]
- Niazi NK, Bashir S, Bibi I, Murtaza B, Shahid M, Javed MT, et al. Phytoremediation of arsenic-contaminated soils using arsenic hyperaccumulating ferns. In: Ansari AA, Singh Gill S, Gill R, Lanza GR, Newman L, editors. Phytoremediation. Berlin: Springer; 2016. [DOI:10.1007/978-3-319-40148-5_19]
- Onyia PC, Ozoko DC, Ifediegwu SI. Phytoremediation of arsenic-contaminated soils by arsenic hyperaccumulating plants in selected areas of Enugu State, Southeastern, Nigeria. Geol Ecol Landsc. 2021; 5(4):308-19. [DOI:10.1080/24749508.2020.1809058]
- Souza TD, Borges AC, Braga AF, Veloso RW, Teixeira de Matos A. Phytoremediation of arsenic-contaminated water by Lemna Valdiviana: An optimization study. Chemosphere. 2019; 234:402-8. [DOI:10.1016/j.chemosphere.2019.06.004] [PMID]
- Jasrotia S, Kansal A, Mehra A. Performance of aquatic plant species for phytoremediation of arsenic-contaminated water. Appl Water Sci. 2017; 7(2):889-96. [DOI:10.1007/s13201-015-0300-4]
- Nazir M, Idrees I, Danish P, Ahmad S, Ali Q, Malik A. Potential of water hyacinth (Eichhornia crassipes L.) for phytoremediation of heavy metals from waste water. Biol Clin Sci Res J. 2020; 2020(1):1-6. [DOI:10.54112/bcsrj.v2020i1.6]
- Ali S, Abbas Z, Rizwan M, Zaheer IE, Yavas I, Unay A, et al. Application of floating aquatic plants in phytoremediation of heavy metals polluted water: A review. Sustainability. 2020; 12(5):1-33. [DOI:10.3390/su12051927]
- Garcia-Salgado S, Garcia-Casillas D, Quijano-Nieto MA, Bonilla-Simon MM. Arsenic and heavy metal uptake and accumulation in native plant species from soils polluted by mining activities. Water Air Soil Pollut. 2012; 223(2):559-72. [DOI:10.1007/s11270-011-0882-x]
- Srivastava M, Ma LQ, Santos JA. Three new arsenic hyperaccumulating ferns. Sci Total Environ. 2006; 364(1-3):24-31. [DOI:10.1016/j.scitotenv.2005.11.002] [PMID]
- Kalve S, Sarangi BK, Pandey RA, Chakrabarti T. Arsenic and chromium hyperaccumulation by an ecotype of Pteris vittata-prospective for phytoextraction from contaminated water and soil. Curr Sci. 2011; 100(6):888-94. [Link]
- Wang J, Feng X, Anderson CW, Xing Y, Shang L. Remediation of mercury contaminated sites-A review. J Hazard Mater. 2012; 221-222:1-18. [DOI:10.1016/j.jhazmat.2012.04.035] [PMID]
- Sas-Nowosielska A, Galimska-Stypa R, Kucharski R, Zielonka U, Małkowski E, Gray L. Remediation aspect of microbial changes of plant rhizosphere in mercury contaminated soil. Environ Monit Assess. 2008; 137(1-3):101-9. [DOI:10.1007/s10661-007-9732-0] [PMID]
- Rodriguez L, Lopez-Bellido FJ, Carnicer A, Alcalde-Morano V. Phytoremediation of mercury-polluted soils using crop plants. Fresenius Environmental Bulletin; 2003. 12(9):967-71. [Link]
- Rodriguez L, Rincón J, Asencio I, Rodríguez-Castellanos L. Capability of selected crop plants for shoot mercury accumulation from polluted soils: Phytoremediation perspectives. Int J Phytoremediation. 2007; 9(1):1-13. [DOI:10.1080/15226510601139359] [PMID]
- Zheng N, Liu J, Wang Q, Liang Z. Mercury contamination due to zinc smelting and chlor-alkali production in NE China. Appl Geochem. 2011; 26:188-93. [DOI:10.1016/j.apgeochem.2010.11.018]
- Chen J, Shiyab S, Han FX, Monts DL, Waggoner CA, Yang Z, et al. Bioaccumulation and physiological effects of mercury in Pteris vittata and Nephrolepis exaltata. Ecotoxicology. 2009; 18(1):110-21. [DOI:10.1007/s10646-008-0264-3] [PMID]
- Wang Y, Stauffer C, Keller C, Greger M. Changes in hg fractionation insoil induced by willow. Plant Soil. 2005; 275:67-75. [DOI:10.1007/s11104-004-6108-x]
- Pérez-Sanz A, Millán R, Sierra MJ, Alarcón R, García P, Gil-Díaz M, et al. Mercury uptake by silene vulgaris grown on contaminated spiked soils. J Environ Manage. 2012; 95 Suppl:S233-7. [DOI:10.1016/j.jenvman.2010.07.018] [PMID]
- Koptsik G. Problems and prospects concerning the phytoremediation of heavy metal polluted soils: A review. Eurasian Soil Sci. 2014; 47:923-39. [DOI:10.1134/S1064229314090075]
- Kucharski R, Sas-Nowosielska A, Małkowski E, Japenga J, Kuperberg JM, Pogrzeba M, et al. The use of indigenous plant species and calcium phosphate for the stabilization of highly metal-polluted sites in southern Poland. Plant and Soil. 2005; 273(1/2):291-305. [DOI:10.1007/s11104-004-8068-6]
- Chehregani A, Malayeri BE. Removal of heavy metals by native accumulator plants. Int J Agric Biol. 2007; 9:462-5. [Link]
- Cunningham SD, Ow DW. Promises and prospects of phytoremediation. Plant Physiol. 1996; 110(3):715-9. [DOI:10.1104/pp.110.3.715] [PMID] [PMCID]
- Rai PK. Phytoremediation of Hg and Cd from industrial effluents using an aquatic free floating macrophyte Azolla pinnata. Int J Phytoremediation. 2008; 10(5):430-9. [DOI:10.1080/15226510802100606] [PMID]
- Wei S, Teixeira da Silva JA, Zhou Q. Agro-improving method of phytoextracting heavy metal contaminated soil. J Hazard Mater. 2008; 150(3):662-8. [DOI:10.1016/j.jhazmat.2007.05.014] [PMID]
- Zhang X, Xia H, Li Z, Zhuang P, Gao B. Identification of a new potential Cd-hyperaccumulator Solanum photeinocarpum by soil seed bank-metal concentration gradient method. J Hazard Mater. 2011; 189(1-2):414-9. [DOI:10.1016/j.jhazmat.2011.02.053] [PMID]
- Li YM, Chaney R, Brewer E, Roseberg R, Angle JS, Baker A, et a. Development of a technology for commercial phytoextraction of nickel: Economic and technical considerations. Plant Soil. 2003; 249:107-15. [DOI:10.1023/A:1022527330401]
- Bani A, Pavlova D, Echevarria G, Mullaj A, Reeves RD, Morel JL, et al. Nickel hyperaccumulation by the species of Alyssum and Thlaspi (Brassicaceae) from the ultramafic soils of the Balkans. Botanica Serbica. 2010; 34:3-14. [Link]
- Prasad MNV. Nickelophilous plants and their significance in phytotechnologies. Braz J Plant Physiol; 2005; 17(1):113-28. [DOI:10.1590/S1677-04202005000100010]
- Mesjasz-Przybylowicz J, Nakonieczny M, Migula P, Augustyniak M, Tarnawska M, Reimold WU, et al. Uptake of cadmium, lead, nickel and zinc from soil and water solutions by the nickel hyperaccumulator Berkheya coddii. Acta Biol Cracov Ser Bot. 2004; 46:75-85. [Link]
- Altinözlü H, Karagöz A, Polat T, Ünver İ. Nickel hyperaccumulation by natural plants in Turkish serpentine soils. Turkish J Bot. 2012; 36(3):269-80. [DOI:10.3906/bot-1101-10]
- Chaney RL, Broadhurst CL, Centofanti T. Phytoremediation of soil trace elements. In: Hooda PS, editor. Trace elements in soils. Hoboken: John Wiley & Sons; 2010. [DOI:10.1002/9781444319477.ch14]
- Jaffré T, Brooks RR, Lee J, Reeves RD. Sebertia acuminata: A hyperaccumulator of nickel from New Caledonia. Science. 1976; 193(4253):579-80. [DOI:10.1126/science.193.4253.579] [PMID]
- Sakakibara M, Ohmori Y, Ha NTH, Sano S, Sera K. Phytoremediation of heavy metal-contaminated water and sediment by Eleocharis acicularis. Clean Soil Air Water. 2011; 39(8):735-41. [DOI:10.1002/clen.201000488]
- Sheoran V, Sheoran AS, Poonia P. Phytomining: A review. Miner Eng. 2009; 22(12):1007-19. [DOI:10.1016/j.mineng.2009.04.001]
- Yang SX, Deng H, Li MS. Manganese uptake and accumulation in a woody hyperaccumulator, Schima superba. Plant Soil Environ. 2008; 54:441-6. [DOI:10.17221/401-PSE]
- Harris AT, Naidoo K, Nokes J, Walker T, Orton F. Indicative assessment of the feasibility of Ni and Au phytomining in Australia. J Clean Prod. 2009; 17(2):194-200. [DOI:10.1016/j.jclepro.2008.04.011]
- Koopmans GF, Romkens PFAM, Song J, Temminghoff EJM, Japenga J. Predicting the phytoextraction duration to remediate heavy metal contaminated soils. Water Air Soil Pollut. 2007; 181:355-71. [DOI:10.1007/s11270-006-9307-7]
- Mendez MO, Maier RM. Phytostabilization of mine tailings in arid and semiarid environments--an emerging remediation technology. Environ Health Perspect. 2008; 116(3):278-83. [DOI:10.1289/ehp.10608] [PMID] [PMCID]
- DalCorso G, Fasani E, Manara A, Visioli G, Furini A. Heavy metal pollutions: state of the art and innovation in phytoremediation. Int J Mol Sci. 2019; 20(14):3412. [DOI:10.3390/ijms20143412] [PMID] [PMCID]
- Heaton ACP, Rugh CL, Wang NJ, Meagher RB. Phytoremediation of mercury- and methylmercury-polluted soils using genetically engineered plants. J Soil Contam. 7(4):497-509. [DOI:10.1080/10588339891334384]
- Moreno FN, Anderson CW, Stewart RB, Robinson BH. Mercury volatilisation and phytoextraction from base-metal mine tailings. Environ Pollut. 2005; 136(2):341-52. [DOI:10.1016/j.envpol.2004.11.020] [PMID]
- Wang M, Chen S, Jia X, Chen L. Concept and types of bioremediation. In: Hasanuzzaman M, Vara Prasad MN, editors. Handbook of bioremediation. Cambridge: Academic Press; 2021. [DOI:10.1016/B978-0-12-819382-2.00001-6]
- Surriya O, Saleem SS, Waqar K, Kazi AG. Phytoremediation of Soils: Prospects and challenges. In: Soil remediation and plants. Cambridge: Academic Press; 2015. [DOI:10.1016/B978-0-12-799937-1.00001-2]
- Rawat K, Fulekar MH, Pathak B. Rhizofiltration: A green technology for remediation of heavy metals. Int J Innov Bio Sci. (2012; 2(4):193-9. [Link]
- Dushenkov S, Vasudev D, Kapulnik Y, Gleba D, Fleisher D, Ting KC, et al. Removal of uranium from water using terrestrial plants. Environ Sci Technol. 1997; 31(12):3468-74. [DOI:10.1021/es970220l]
- Ma Y, Prasad MN, Rajkumar M, Freitas H. Plant growth promoting rhizobacteria and endophytes accelerate phytoremediation of metalliferous soils. Biotechnol Adv. 2011; 29(2):248-58. [DOI:10.1016/j.biotechadv.2010.12.001] [PMID]
- Spaepen S, Vanderleyden J. Auxin and plant-microbe interactions. Cold Spring Harb Perspect Biol. 2011; 3(4):a001438. [DOI:10.1101/cshperspect.a001438] [PMID] [PMCID]
- Göhre V, Paszkowski U. Contribution of the arbuscular mycorrhizal symbiosis to heavy metal phytoremediation. Planta. 2006; 223(6):1115-22. [DOI:10.1007/s00425-006-0225-0] [PMID]