Introduction
Diabetes has been recognized as an illness by ancient Egyptian and Indian physicians who identified the disease as “too great emptying of urine.” The ancient Indian physicians Charak and Sushruta described different symptoms of the disease, which were later classified as type I and type II diabetes [
1]; However, its course and cure were not sufficiently investigated. Considering the level of scientific knowledge of that period, this issue was somewhat expected. In the past few decades, the world has seen an explosion in diabetes and its related serious complications [
2-
4]. Regarding mortality, diabetes occupies a position in the top 10 diseases. This resulted in concerted attempts to cure diabetes.
Since 2000, the International Diabetes Federation has been reporting the regional and global occurrence of diabetes. As per their report, the disease is progressing almost exponentially. In 2019, approximately 463 million people suffered from the disease, which had grown to 537 million adults by 2021 [
5]. The number is projected to reach 643 million by 2030 and 783 million by 2045 [
6]. Of the two types, type 1 diabetes is considered an incurable disease and is managed by insulin supplementation. In addition to the pain and anxiety, the treatment is financially challenging as well. In 2017, global health expenditure due to diabetes treatment was estimated at USD 727 billion and is projected to reach USD 825 billion by 2030 [
7].
In the absence of a cure, exogenous insulin treatment has remained a mainstay in treating severe cases. Still, it can result in episodes of hyperglycemia and hypoglycemia if not monitored religiously [
8].
Of late, the focus of diabetes research has shifted from management to cure. With the advent of tissue regenerative technologies, there is a new hope to replace the damaged β cells with healthy ones to receive a cure. This review gives an overview of various research strategies used to replace or regenerate β cells for curing diabetes.
Strategies used for β cell supplementation
Transplantation of the whole organ
The effort to cure type 1 diabetes through transplantation of the whole pancreas started quite early. The first attempt to cure diabetes by grafting pancreatic tissue into the human body was made by Dr. Watson Williams in 1893. Dr. Willams grafted three fragments of sheep pancreas into the subcutaneous tissue of a 15-year-old diabetic patient [
9]. This study aims to reestablish normoglycemia by replenishing the depleted pancreatic islets [
10]. The first attempt to replace diseased pancreas with healthy human tissue (duct ligated segmental pancreas) was made in 1966 at the University of Minnesota, Minneapolis [
11,
12]. The procedure was investigated and modified for the next two decades to increase efficiency.
Pancreas transplantation, using allogeneic organs procured from deceased multi-organ donors, was a surgical procedure that was met with success [
13]. However, not every diabetic patient could withstand the rigors of this procedure. Patients who have type 1 diabetes along with healthy and free of secondary complications (body mass index [BMI] <30 kg/m2) were considered suitable for the procedure [
14]. The legal procedure and the availability of matching donors was a constraint. There was some inherent difficulty associated with the process. In addition to the common risk of major surgery, there was a need for long-term immunosuppression [
15]. Moreover, the risk of death due to the failure of the process was relatively high when the pancreas was transplanted alone. As the pancreas shares blood supply with other organs (liver and pancreas), simultaneous transplantation of both organs lowered the risk significantly [
16].
However, the progress was less than satisfactory when long-term survival was considered. The graft loss within the first 90 days of the transplantation was one significant difficulty with the procedure. Although the rejection rate declined from 14% in 1999 to 8.2% in 2014, rather than the whole pancreas, grafting a cluster of insulin-producing cells would be a better alternative [
17].
β cell transplantation
In humans, insulin is produced in the pancreatic islet, which houses mainly three types of cells (α, β, γ). Of these, only β cells produce insulin while α cell releases glucagon, which helps convert glycogen to free glucose to counteract hypoglycemia. Each islet carries approximately 1560±20 cells, most of which are β-cells (1140±15) [
18]. Volume-wise, islet occupies only 2% of the whole pancreas, and supplying nutrition to this small volume of cells is considered far more convenient than supporting the entire pancreas. An experiment by Lacy and Cols in 1972 brought the idea to the forefront [
19]. The researchers transplanted the diabetic rodents with pancreatic islets and successfully reversed the hyperglycemia. Henceforth, the focus for curing type 1 diabetes was directed to autologous islet transplantation [
20]. The procedure came with an added advantage. There was no restriction for placing the islet close to its normal position in the body. Any body area with a good nutrient and oxygen supply could be used to support the insulin-producing cells. However, separating the islets from the whole pancreas is time-consuming and laborious [
21].
The challenges and the following issues needed consideration to make the treatment successful.
Donors
Although the transplantation of healthy β cells is the perfect fix for treating severe type 1 diabetes, the process is highly complicated. For insulin-independent survival, greater than 5000 islets/kg of body weight should be grafted in a human [
22]. The availability of the right kind of islet is a challenge, and often, allogenic transplantation is the only way to supply the need. The introduction of the Edmonton protocol brought hope as the pancreas extracted from diseased people could be used as transplants [
23]. However, the shortage of pancreas and the legalities involved with the deceased donors remain one of the bottlenecks. The problem gets multiplied by the low extraction yield of islets as well. A sizeable fraction of islets are lost during the process (extraction and intra-portal islet infusion) [
22].
Harvesting
In the extraction phase, tissue is cut into small pieces using surgical scissors, and cells are extracted by collagenase treatment. Within the islets, most cells are β cells; however, they also stay in association with other cells. In reality, recipients receive a large volume of islets (11000 islet equivalents per kg of the body weight) extracted from two or more donors for successful transplantation [
24]. Meanwhile, β cells start dying fast once the blood circulation stops. This translates to a greater demand for islets and an increased risk of graft dysfunction [
25].
Transplantation
In the sequence of events, the actual transplantation is the least difficult. The purified β cells are slowly infused into the portal vein of patients through a catheter. The cells settle in the liver. With its rich nutrient and oxygen supply, the liver plays home to the β cells that produce insulin to affect normoglycemia. However, the real challenge comes after the transplantation.
Protecting the transplanted cells
In the human body, β-cell development occurs during the first trimester of pregnancy. The proliferation of β cells continues in the postnatal period as well; however, the rate of cell mitosis declines progressively. A stable state is reached in the second year of life [
26]. After transplantation, islet functions are gradually lost for various reasons, such as the quality of islets used and the alloimmune response [
27]. Several strategies have been tried to protect and keep the transplanted beta cells functional.
Immunosuppression
Transplanted β cells are a target for allograft rejection, and in the absence of immunosuppression, they cannot survive long. Lymphocytes infiltrate the allogeneic islets, leading to islet-specific antibody production, making using immunosuppressants necessary [
28, 29]. In type 1 diabetes, β cells are mainly attacked by T cells, and immunosuppressive agents prevent T-cell clonal expansion.
Immunosuppression can be done in various ways. Two crucial mechanisms by which these agents work include T-cell and B-cell targeting therapy. Therapeutic agents that target T cell function can be broadly categorized based on two types of signals they inhibit. Drugs like cyclosporine and tacrolimus are calcineurin inhibitors (signal 1). They disrupt the calcineurin-dependent signaling pathway, leading to initial T cell gene transcription necessary for additional activation. On the other hand, abatacept and belatacept are signal two targeting agents. Rituximab, ocrelizumab, ofatumumab, and veltuzumab are the agents that target the B-cell. The goals of B cell inhibition include inhibiting the humoral response to auto- or alloantigen, antigen presenting cell function, and B/T cell interactions that lead to efficient T cell activation and proliferation. Immunosuppressive therapy comes with several side effects. Some of calcineurin inhibitors’ most common side effects are nephrotoxicity, electrolyte disturbances (hyperkalemia and hypomagnesemia), hypertension, and neurotoxicity (manifesting as tremor or headache). Signal 2 inhibitors cause headache, nausea, or cold symptoms, such as stuffy head or nose tic. Pain, irritation, or swelling at or near the injection site are common occurrences with both types. Sometimes, the side effects are more harmful than the disease they aim to cure [
30].
Creating a barrier around the β cells
Physical separation was the second strategy to protect the β cells from immune attack. If the islets could be covered with a semipermeable film, it might initially obstruct the recognition process and stop the cascade of immunological events. In such cases, immune suppressants will not be needed. The idea soon caught up with the researchers and developed into a major field in beta cell transplantation research [
31]. Normally, cells produce proteins, approximately 50% of which get converted into particulate insulin. Once formed, this very particle takes part in regulating β cell activities [
32].
Wrapping of β cells within a membrane imposes additional demand on the system. The membrane should be inert and compatible with its capsulated contents to prevent immune rejection. It should be able to maintain its physical integrity and provide a smooth surface so that protein and cell attachment is prevented [
33]. Finally, there is a need to develop blood vessels around the encapsulated islets to ensure cell survival immediately after the process of transplantation [
32].
Hydrogel-coated β cells
Hydrogels are networks of polymeric materials that, because of their structure, can imbibe high quantities of water but do not dissolve in it [
30]. Due to their similarity with the extracellular environment, they provide a friendly atmosphere for the cells to survive and grow [
34]. Many factors must be considered for creating a coating membrane around the β islets. First, in addition to being biocompatible, it should be able to create a physical barrier to conceal the surface of transplanted cells. Secondly, it should allow the passage of nutrients and oxygen but prevent cytotoxic substances from accessing the cell [
30]. Few materials can fulfill this demand (for instance, the hydrogel can satisfy this demand). In short, the membrane should be semi-permeable, selectively allowing only the nutrients inside and the end products of metabolism of the islet cell outside. This property highly depends on the membrane’s pore size, thickness, and rigidity. Any foreign body would attract anti-inflammatory cells [
35], which can induce graft rejection through the local anti-inflammatory reaction. The attachment of the inflammatory cells around the membrane may result in an impermeable fibrotic capsule, starving the inner resident cells of oxygen and nutrients [
36, 37]. Therefore, the selection of membrane material is a crucial factor for the survival of the graft.
Both natural (alginate, chitosan, agarose, fibrin) and synthetic polyethylene glycol (PEG) hydrogels are used for islet encapsulation [
37]. Researchers especially consider PEG favorable as they show better tunability than the natural types in terms of porosity, stability, biocompatibility, and mechanical strength. The mechanical properties of the PEG-based hydrogels can be tuned by altering concentration and molecular weight. The stiffness of hydrogel is an essential criterion in islet transplantation as soft gels cause less anti-inflammatory reaction compared to stiffer gels. At a concentration level of 5% to 10%, PEG generates a soft hydrogel [
38].
Cell survival was also affected by the chemical composition of the encapsulating material. Islets coated with polyethylene di-acrylate, when embedded in a complex hydrogel of thio-glycosaminoglycan, thiogelatin, and thioheparin, could maintain the structure and function of islets and improve the formation of blood vessels. Co-encapsulation of immunomodulators has also been investigated [
32].
Capsules containing PEG on the surface are also shown to reduce the secretion of interleukin-2 – a cytokine produced by the T lymphocytes. IL-2 affects graft rejection by supporting other T and B lymphocytes’ growth and activity [
32].
Because of the apparent advantages of the encapsulation technique, in-depth research has been done on this aspect. Encapsulation of the β cells by hydrogel can be done in many ways, which include: A) Nanoencapsulation, by placing thin hydrogel films around individual islets, B) Microencapsulation of small groups of islets, individual islets, or other insulin-producing cells within spherically shaped hydrogel microcapsules, C) Macro-encapsulation of islets or other insulin-producing cells within bulk hydrogels that can be shaped and molded within encapsulating devices [
39].
Though islet encapsulation is a great technique to isolate the β cells from physical interaction with the immune system, the method has several difficulties. Too many factors need to be controlled in this method. The nature of the polymer that makes the membrane, its pore size, and its thickness affect the mass exchange between the cells and their environment. Often, cell survival is reduced by hypoxia and insufficient nutrients. PEG-based hydrogels suffer from the drawback of being hydrolytically degradable [
30], and the protection offered by the polymeric membrane is limited by its degradation time. With time, hydrogels the encapsulating media, degrade, and islets are exposed to the immune attack. The process is slow and fails to provide permanent insulin independence. Currently, several clinical trials are going on to assess the success of this technique.
Table 1 mentions some of the important studies [
40].

3D bioprinting
3D bioprinting is a novel technology that can construct any tissue starting from scratch. To keep the cells alive and functional, they are suspended in a matrix that resembles the natural matrix of the body. Since hydrogels resemble the body matrix in many ways, cells are usually suspended in hydrogels. The combination of cell and hydrogel is known as bioink. In this technique, bioink is laid layer by layer on a surface to create 3-dimensional shapes.
Polymers are the backbone of hydrogels, and natural polymers, because of their excellent cytocompatibility, are preferred in making the bioink. As the medium is aqueous, substances required for cell growth (growth factor and other bioactive agents) can be co-administered. For ease of spraying, the material should be fluid; however, the fluidity must be reduced once sprayed. Without solidification, the shape cannot be retained. The conversion of the bioink from a fluid to a solid can be done in several ways. The exact mechanism depends upon the nature of the hydrogel medium. It is often done using bioinks with cross-linking agents dissolved in them.
Mechanism-wise, bioprinting has evolved into four major types as follows: Extrusion-based, inkjet-based, stereo-lithography-based, and laser-energy-driven. Solid structures can be created by using the VAT polymerization technique. Accordingly, a polymeric bioink (photopolymer) containing live cells is deposited layer by layer on a predetermined design. As this arrangement is exposed to light of appropriate frequency, the resin solidifies or cures into the desired shape.
Figure 1 depicts different bioprinting approaches for creating pancreatic constructs [
41].
In all forms, bioink is deposited by gentle force at a specific design generated by a computer. The deposition is done through a nozzle or as a mist in a controlled manner. Though the technique has created much hype, the concept’s commercial viability is faced with several challenges. Both cell survival and functionality are affected by the stress imposed by the printing process. For example, in inkjet- and extrusion-based bioprinting, cells are subjected to considerable shear stress during ejection. In laser-driven and stereo-lithography-based bioprinting, cells endure thermal and radiative stress. Cell viability is also affected by changes in temperature rheological properties of hydrogels. This stress often results in cell shrinkage and condensation of nuclei, which compromise their functionality even if they survive.
The attempt to create a bioartificial pancreas by 3D printing technology was first reported by Professor Wang in 2009 [
42]. In a medium of natural hydrogels, Professor Wang had embedded adipose stem cells. When these embedded cells and islets were printed on the live organs of similar physiological characteristics, adipose stem cells differentiated into vascular stem cells and adipocytes. When different drugs challenged the system, they showed the standard physiological response.
The work was a significant contribution to the development of strategies to cure type 1 diabetes. Later, another group of researchers encapsulated the islets into alginate/methyl cellulose hydrogels and formed a 3D structure by bio-printing. Within the hydrogel covering, islets functioned efficiently to produce insulin and glucagon [
43].
For efficient printing, bioink should have good flowability. A research group on this aspect produced new media for bioink by incorporating Pluronic F127 and hypomethylated pectin into the alginate solution. Pluronic F 127 is a polymeric surfactant from ethylene and polypropylene. Aqueous Pluronic F 127 solutions are plastic and have a high yield stress value [
44,
45]. The process of bioprinting imposes significant stress on the cells.
Creating insulin-producing cells from stem cells
Attention has recently been drawn to producing insulin-secreting cells by differentiating stem cells. Stem cells obtained from various sites are being studied. Stem cells from the umbilical cord are highly efficient in differentiating into insulin-producing cells.
Perhaps the most promising source of beta cells is the inner cell mass of blastocysts of human embryos [
46]. Extensive research is being conducted to produce β and β progenitor cells from mesenchymal stem cells (MSC) [
47]. MSC cells release immunomodulatory molecules, which can prevent β-cell destruction [
48]. However, removing the stem cells from live donors is a big hurdle. A renewable source of the same could be deceased donors, though the process is elaborate [
49].
Isolation of mesenchymal stem cells
Rao et al [
49] described a method for the preparation of beta cells using mesenchymal stem cells of deceased donors. Adipose tissue obtained from the abdomen was minced into pieces and suspended in 0.9% saline, which was filtered through a series of bags (AC: Px) and centrifuged to obtain vascular stromal fraction. The cell pellet contained MSCs and other cell types identified through phenotyping by flow cytometric analysis.
Expansion of mesenchymal stem cells
Cells (after proper counting) were added to the conditioned cell culture flask using special media that supported the growth of MSC. Once 80% confluency was obtained, they were removed by trypsinization procedure for further passaging. MSCs are made to differentiate into β cells through the following three-step process.
Pancreatic cells have their origin in the endodermal germ layer. Hence, the first stage in converting MSCs to β progenitor cells is the formation of definitive endoderm- a transient phase in which an epidermal layer is created. MSCs and other growth factors are grown for two days in serum-free DMEM/F12.
In the second phase, the cells are suitably diluted to a desired cell concentration in CTS (TM) media and added to culture plates. A complete growth media (DMEM/F12) is added as the cells settle down at the surface. In this phase, cells are induced to differentiate into pancreatic endoderm using another special medium. The duration of this stage is approximately two days.
MSCs are finally converted to β cells in the third stage using a glucagon-like peptide -1, containing growth media. Glucagon-like peptide -1 promotes β-cell survival [
50]. The conversion to β cells is confirmed by immunohistochemistry.
ViaCyte, a San Diego based company, is much in the news for developing two products, PEC direct and PEC encap, which can potentially mimic the pattern of real-time blood glucose level regulation. Both systems use stem cells to regenerate pancreatic islet progenitor cells known as PEC-01™ cells. In humans with functional pancreas, the glucose level is maintained at a physiologically normal level mainly by both α and β cells. The PEC-01™ cells can differentiate into β and α cells and are especially recommended for type 1 diabetic patients suffering from frequent hypoglycemia. In the PEC direct system, the cells are enclosed in a perforated pouch, which allows direct vascularisation. In contrast, the PEC encap has a membrane wrapping around it to protect the progenitor cells from the recipient’s immune system to minimize the need for immunosuppression. 2014, they got Food and Drug Administration (FDA) approval for conducting clinical trials. The results of the test were mixed. The cell survival was prolonged up to 24 months but highly variable among subjects. Foreign body reaction to the device components is suspected to be the cause [
51].
Figure 2 explains the development method of the PEC-Encap device created by ViaCyte.
Conclusion
Curing type 1 diabetes by replacing the insulin-deficient pancreas with a healthy one is not a new idea. The first transplantation occurred as early as 1966, and the process was met with reasonable success. The chief advantage associated with this method was that insulin-producing islet cells were present in their natural environment. According to the international pancreas transplant registry reports, over 67000 transplants have been performed globally; however, the major disadvantage associated with this procedure is invasiveness and strong immunogenicity. Moreover, the demand for the pancreas outnumbers supply. The majority of pancreas grafts are retrieved from brain-dead donors whose pancreatic cells are still alive. The process is subjected to strict legal procedures and narrow acceptance criteria (BMI, age, lifestyle factors). Regardless of these constraints, it is the best short and long-term treatment to achieve insulin independence for type 1 diabetic patients.
In the next phase, the research shifted from organ to islet transplantation. As islets comprise 1% to 2% of the pancreas, the transplantation could be achieved through a minimally invasive process. However, there is a significant challenge regarding the survival of islets. Cells need a natural microenvironment to stay functional, but direct transplantation exposes them to immunological attack. Hydrogels, with their tissue-like properties, showed the promise of being a natural barrier. Hence, extensive research was undertaken to encapsulate the islets in hydrogels. Yet this manipulation was not foolproof. Even a fully protected β cell cannot keep the insulin level normal. In a healthy individual, insulin production is regulated by β cells only. Meanwhile, α, somatostatin, and ghrelin cells also significantly influence this process. Bioprinting, which can create mini-organs comprising different kinds of cells, mimicking their natural orientation, was thought to resolve this problem. However, this technique is still in its infancy and is likely to need considerable research investment before it finds a place in regular clinical practice.
The percentage of cell survival improved. The process of transplantation was minimally invasive. Moreover, allogeneic islets could reduce the gap between supply and demand. However, the efficiency of the process is less than desirable.
With the advances in stem cell research, another promising avenue for achieving insulin independence has opened the production of insulin-producing cells from stem cells. In this strategy, insulin-producing cells are biotechnologically expanded to transplantation quality β cells. Stem cells produce the supporting cells, which promote insulin homeostasis. However, this technique requires a high volume of cells per patient because of the exponential post-transplantation loss of these cells. Another major risk of using progenitor cells is neoplasia. Considering these risks, it is highly likely that progenitor cells will remain experimental for some more time until the pros and cons of the technique are properly studied. At present, extensive clinical trials are on to assess the technique’s efficacy (
Table 2).
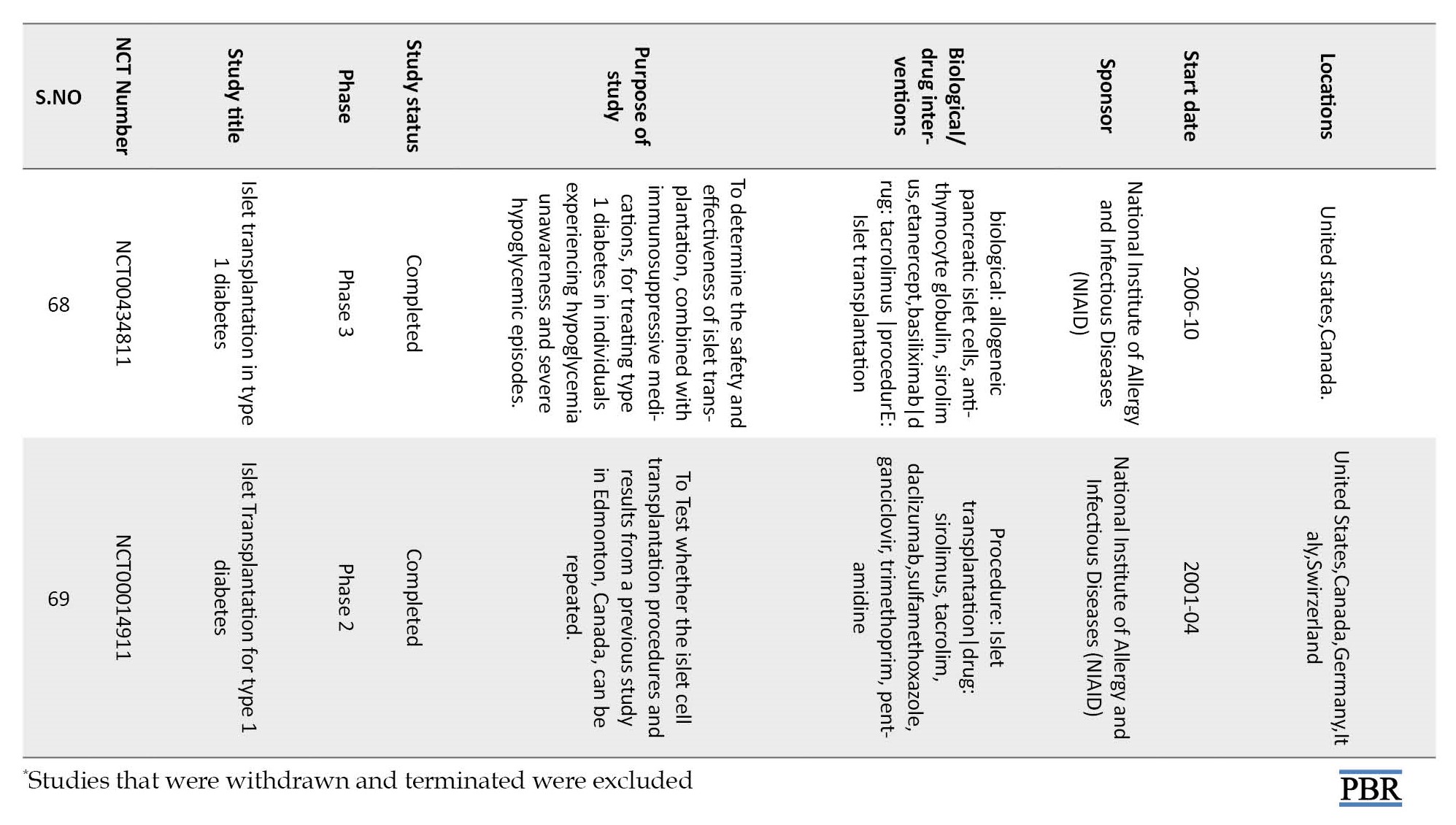
The critical question, “which is a better technique, islet transplantation or stem cell therapy?” is still unanswered. Both methods, bioprinting and stem cell therapy, show the prospect of a lasting cure, and at the current level, both are extremely expensive. A technique that introduces a safer product into the body will likely be more favorable, and cost will be one of the deciding factors.
Ethical Considerations
Compliance with ethical guidelines
There were no ethical considerations to be considered in this review.
Funding
This research did not receive any grant from funding agencies in the public, commercial, or non-profit sectors.
Authors' contributions
All authors contributed equally to preparing this review.
Conflict of interest
The authors declared no conflict of interest.
Acknowledgments
The authors acknowledge Subhadip Das, for his help during the preparation of manuscript and Subhodip Debnath, NSHM Knowledge Campus for drawing the figures.
References
- Lakhtakia R. The history of diabetes mellitus. Sultan Qaboos Univ Med J. 2013; 13(3):368-70. [DOI:10.12816/0003257] [PMID]
- Roglic G. WHO Global report on diabetes. Int J Noncommun Dis. 2016; 1(1):3-8. [DOI:10.4103/2468-8827.184853]
- Cooke DW, Plotnick L.Type 1 diabetes mellitus in pediatrics. Pediatr Rev. 2008; 29(11):374-84; quiz 385. [DOI:10.1542/pir.29-11-374] [PMID]
- Harlan DM, Kenyon NS, Korsgren O, Roep BO; Immunology of Diabetes Society. Current advances and travails in islet transplantation. Diabetes. 2009; 58(10):2175-84. [DOI:10.2337/db09-0476] [PMID]
- Saeedi P, Petersohn I, Salpea P, Malanda B, Karuranga S, Unwin N, et al. Global and regional diabetes prevalence estimates for 2019 and projections for 2030 and 2045: Results from the International Diabetes Federation Diabetes Atlas, 9th edition. Diabetes Res Clin Pract. 2019; 157:107843.[DOI:10.1016/j.diabres.2019.107843] [PMID]
- International Diabetes Federation. Facts & figures. Brussels: International Diabetes Federation; 2023. [Link]
- Williams R, Karuranga S, Malanda B, Saeedi P, Basit A, Besançon S, et al. Global and regional estimates and projections of diabetes-related health expenditure: Results from the International Diabetes Federation Diabetes Atlas, 9th edition. Diabetes Res Clin Pract. 2020; 162:108072. [DOI:10.1016/j.diabres.2020.108072] [PMID]
- Espona-Noguera A, Ciriza J, Cañibano-Hernández A, Orive G, Hernández RMM, Saenz Del Burgo L, et al. Review of advanced hydrogel-based cell encapsulation systems for insulin delivery in type 1 diabetes mellitus. Pharmaceutics. 2019; 11(11):597. [DOI:10.3390/pharmaceutics11110597] [PMID]
- Vecchio I, Tornali C, Bragazzi NL, Martini M. The discovery of insulin: An important milestone in the history of medicine. Front Endocrinol (Lausanne). 2018; 9:613. [DOI:10.3389/fendo.2018.00613] [PMID]
- Sutherland DE, Gruessner RW, Dunn DL, Matas AJ, Humar A, Kandaswamy R, et al. Lessons learned from more than 1,000 pancreas transplants at a single institution. Ann. Surg. 2001; 233(4):463-501. [DOI:10.1097/00000658-200104000-00003] [PMID]
- Kelly WD, Lillehei RC, Merkel FK, Idezuki Y, Goetz FC. Allotransplantation of the pancreas and duodenum along with the kidney in diabetic nephropathy. Surgery. 1967; 61(6):827-37. [PMID]
- Squifflet JP, Gruessner RWG, Sutherland DE. The history of pancreas transplantation: Past, present and future. Acta Chir Belg. 2008; 108(3):367-78. [DOI:10.1080/00015458.2008.11680243] [PMID]
- Niclauss N, Meier R, Bédat B, Berishvili E, Berney T. Beta-cell replacement: Pancreas and Islet Cell transplantation. Endocr Dev. 2016; 31:146-62. [DOI:10.1159/000439412] [PMID]
- Aref A, Zayan T, Pararajasingam R, Sharma A, Halawa A. Pancreatic transplantation: Brief review of the current evidence. World J Transplant. 2019; 9(4):81-93. [DOI:10.5500/wjt.v9.i4.81] [PMID]
- Beck J, Angus R, Madsen B, Britt D, Vernon B, Nguyen KT. Islet encapsulation: Strategies to enhance islet cell functions. Tissue Eng. 2007; 13(3):589-99. [DOI:10.1089/ten.2006.0183] [PMID]
- Gruessner AC. 2011 Update on pancreas transplantation: Comprehensive trend analysis of 25,000 cases followed up over the course of twenty-four years at the international Pancreas Transplant Registry (IPTR). Rev Diabet Stud. 2011; 8(1):6-16. [DOI:10.1900/RDS.2011.8.6] [PMID]
- Kandaswamy R, Skeans MA, Gustafson SK, Carrico RJ, Prentice MA, Israni AK, et al. Pancreas. Am j Transplant. 2016; 16 (Suppl 2):47-68. [DOI:10.1111/ajt.13667] [PMID]
- Figliuzzi M, Bonandrini B, Silvani S, Remuzzi A. Mesenchymal stem cells help pancreatic islet transplantation to control type 1 diabetes. World J Stem Cells. 2014; 6(2):163-72.[DOI:10.4252/wjsc.v6.i2.163] [PMID]
- Lacy PE, Walker MM, Fink CJ. Perfusion of isolated rat islets in vitro. Participation of the microtubular system in the biphasic release of insulin. Diabetes. 1972; 21(10):987-98. [DOI:10.2337/diab.21.10.987] [PMID]
- Najarian JS, Sutherland DE, Baumgartner D, Burke B, Rynasiewicz JJ, Matas AJ, et al. Total or near total pancreatectomy and islet autotransplantation for treatment of chronic pancreatitis. Ann Surg. 1980; 192(4):526-42. [DOI:10.1097/00000658-198010000-00011] [PMID]
- Kawahara T, Kin T, Kashkoush S, Gala-Lopez B, Bigam DL, Kneteman NM, et al. Portal vein thrombosis is a potentially preventable complication in clinical islet transplantation. Am J Transplant. 2011; 11(12):2700-7. [DOI:10.1111/j.1600-6143.2011.03717.x] [PMID]
- Shapiro AM. Islet transplantation in type 1 diabetes: Ongoing challenges, refined procedures, and long-term outcome. Rev Diabet Stud. 2012; 9(4):385-406. [DOI:10.1900/RDS.2012.9.385] [PMID]
- Emamaullee JA, Pepper A, Shapiro AMJ. Chapter 56 - Islet cell transplantation. In: Atala A, Lanza R, Mikos AG, Nerem R, editors. Principles of regenerative medicine. Boston: Academic Press; 2019. [DOI:10.1016/B978-0-12-809880-6.00056-4]
- Bux Rodeman K, Hatipoglu B. Beta-cell therapies for type 1 diabetes: Transplants and bionics. Cleve Clin J Med. 2018; 85(12):931-7. [DOI:10.3949/ccjm.85a.17088] [PMID]
- De Vos P, Wolters GH, Fritschy WM, Van Schilfgaarde R. Obstacles in the application of microencapsulation in islet transplantation. Int J Artif Organs. 1993; 16:205-12. [DOI:10.1177/039139889301600407] [PMID]
- Basile G, Kulkarni RN, Morgan NG. How, when, and where do human β-Cells regenerate? Curr Diab Rep. 2019; 19(8):48. [DOI:10.1007/s11892-019-1176-8] [PMID]
- Rother KI, Harlan DM. Challenges facing islet transplantation for the treatment of type 1 diabetes mellitus. J Clin Invest. 2004; 114(7):877-83. [DOI:10.1172/JCI200423235]
- Rodriguez-Calvo T, Ekwall O, Amirian N, Zapardiel-Gonzalo J, von Herrath MG. Increased immune cell infiltration of the exocrine pancreas: a possible contribution to the pathogenesis of type 1 diabetes. Diabetes. 2014; 63(11):3880-90. [DOI:10.2337/db14-0549] [PMID]
- Burrack AL, Martinov T, Fife BT. T cell-mediated Beta cell destruction: Autoimmunity and alloimmunity in the context of type 1 diabetes. Front Endocrinol (Lausanne). 2017; 8:343. [DOI:10.3389/fendo.2017.00343] [PMID]
- David A, Day J, Shikanov A. Immunoisolation to prevent tissue graft rejection: Current knowledge and future use. Exp Biol Med (Maywood). 2016; 241(9):955-61. [DOI:10.1177/1535370216647129] [PMID]
- Schweicher J, Nyitray C, Desai TA. Membranes to achieve immunoprotection of transplanted islets. Front Biosci (Landmark Ed). 2014; 19(1):49-76. [DOI:10.2741/4195] [PMID]
- Xu Y, Song D, Wang X. 3D Bioprinting for pancreas engineering/manufacturing. Polymers. 2022; 14(23):5143. [DOI:10.3390/polym14235143] [PMID]
- Borg DJ, Bonifacio E. The use of biomaterials in islet transplantation.Curr Diab Rep. 2011; ;11(5):434-44. [DOI:10.1007/s11892-011-0210-2] [PMID]
- Guiseppi-Elie A. Electroconductive hydrogels: Synthesis, characterization, and biomedical applications. Biomaterials. 2010; 31(10):2701-16. [DOI:10.1016/j.biomaterials.2009.12.052] [PMID]
- Santos E, Zarate J, Orive G, Hernandez RM, Pedraz JL. Biomaterials in cell microencapsulation. Adv Exp Med Biol. 2010; 670:5-21. [DOI:10.1007/978-1-4419-5786-3_2] [PMID]
- Knobeloch T, Abadi SEM, Bruns J, Zustiak SP, Kwon G. Injectable Polyethylene Glycol Hydrogel for Islet Encapsulation: An in vitro and in vivo Characterization. Biomed Phys Eng Express. 2017; 3:035022. [DOI:10.1088/2057-1976/aa742b] [PMID]
- Espona-Noguera A, Ciriza J, Cañibano-Hernández A, Orive G, Hernández RMM, Saenz Del Burgo L, et al. Review of advanced hydrogel-based cell encapsulation systems for insulin delivery in type 1 diabetes mellitus. Pharmaceutics. 2019; 11(11):597. [DOI:10.3390/pharmaceutics11110597] [PMID]
- Lust ST, Hoogland D, Norman MDA, Kerins C, Omar J, Jowett GM, et al. Selectively cross-linked tetra-PEG hydrogels provide control over mechanical strength with minimal impact on diffusivity. ACS Biomater Sci Eng. 2021; 7(9):4293-304. [DOI:10.1021/acsbiomaterials.0c01723] [PMID]
- Zamboni F, Collins MN. Cell-based therapeutics in type 1 diabetes mellitus. Int J Pharmaceutics. 2017; 521(1-2):346-56. [DOI:10.1016/j.ijpharm.2017.02.063] [PMID]
- United States National Library of Medicine. Publicly accessible registry and results database of clinical trials, Govt of United States [Internet]. 2023 [Updated 19 September 2023]. Available from: [Link]
- Parvaneh S, Kemény L, Ghaffarinia A, Yarani R, Veréb Z. Three-dimensional bioprinting of functional β-islet-like constructs. Int J Bioprint. 2023; 9(2):665. [DOI:10.18063/ijb.v9i2.665] [PMID]
- Xu M, Yan Y, Liu H, Yao Y, Wang X. Control adipose-derived stromal cells differentiation into adipose and endothelial cells in a 3D structure established by cell-assembly technique. J. Bioact Compat Polym. 2009; 24(1_suppl):31-47.[DOI:10.1177/0883911509102794]
- Duin S, Schütz K, Ahlfeld T, Lehmann S, Lode A, Ludwig B, et al. 3D bioprinting of functional islets of langerhans in an alginate/methylcellulose hydrogel blend. Adv Healthc Mater. 2019; 8(7):e1801631. [DOI:10.1002/adhm.201801631] [PMID]
- Shriky B, Kelly A, Isreb M, Babenko M, Mahmoudi N, Rogers S, et al. Pluronic F127 thermosensitive injectable smart hydrogels for controlled drug delivery system development. J Colloid Interface Sci. 2020; 565:119-30. [DOI:10.1016/j.jcis.2019.12.096] [PMID]
- Jalaal M, Cottrell G, Balmforth N, Stoeber B. On the rheology of Pluronic F127 aqueous solutions. J Rheol. 2017; 61:139-46. [DOI:10.1122/1.4971992]
- Hebrok M. Generating β cells from stem cells- story so far. Cold Spring Harb Perspect Med. 2012; 2(6):a007674. [DOI:10.1101/cshperspect.a007674] [PMID]
- Ghoneim MA, Refaie AF, Elbassiouny BL, Gabr MM, Zakaria MM. From mesenchymal stromal/stem cells to insulin-producing cells: Progress and challenges. Stem Cell Rev Rep. 2020; 16(6):1156-72. [DOI:10.1007/s12015-020-10036-3] [PMID]
- Silva IBB, Kimura CH, Colantoni VP, Sogayar MC. Stem cells differentiation into insulin-producing cells (IPCs): Recent advances and current challenges. Stem Cell Res Ther. 2022; 13(1):520. [DOI:10.1186/s13287-022-03206-2] [PMID]
- Rao P, Deo D, Marchioni M. Differentiation of human deceased donor, adipose-derived, mesenchymal stem cells into functional Beta Cells. J Stem Cells Regen Med. 2020; 16(2):63-72. [DOI:10.46582/jsrm.1602010] [PMID]
- Linnemann AK, Blumer J, Marasco MR, Battiola TJ, Umhoefer HM, Han JY, et al. Interleukin 6 protects pancreatic β cells from apoptosis by stimulation of autophagy. FASEB J. 2017 ; 31(9):4140-52. [DOI:10.1096/fj.201700061RR] [PMID]
- Cooper-Jones B, Ford C. Islet cell replacement therapy for insulin-dependent diabetes. 2017 Jun 1. In: CADTH issues in emerging health technologies. Ottawa (ON): Canadian Agency for Drugs and Technologies in Health; 2016–2021. 157. [PMID]